Phillip Antunes – Team Mixing Technologies Inc
ABSTRACT
While fly ash is often used as a partial substitute for cement in civil construction, its usage in two-component grouts for TBM annulus grouting is varied. Opinions on the benefits of fly ash vary from person to person and project to project as do the economics. While most tunnelling projects use fly ash in two-component grouts a significant number of projects do not. The purpose of this paper is to quantify the performance of fly ash in two-component grouts by testing the compressive strength, bleed, gel time, and viscosity and also comparing these same parameters with fly ash from different geographic sources.
INTRODUCTION
The use of natural pozzolans predates modern Portland cement by about 2,000 years. Numerous structures built using volcanic ash—a pozzolan—mixed with burned lime are still standing today. In fact, the term pozzolan is derived from the volcanic ash mined at Pozzuloli, a village near Naples, Italy, following the 79 ad eruption of Mount Vesuvius (Derucher et al., 1994). It is not surprising then to see pozzolans, though predominately now synthetic, still in high demand today.
A pozzolan, by definition, is a “siliceous or aluminosiliceous material which in itself possesses little or no cementitious value but will, in finely divided form and in the pres- ence of moisture, chemically react with calcium hydroxide at ordinary temperatures to form compounds possessing cementitious properties” (Derucher et al., 1994). In short, pozzolans are powders that will harden when combined with lime and water at normal temperatures.
Fly ash, the most widely used pozzolan in concrete, is a by-product from the combustion of pulverized coal in electric power generating plants. Upon ignition, most of the volatile matter and carbon in the coal are burned off. However, the coal’s mineral impurities either settle to the furnace bottom as bottom ash or fuse in suspension and are carried away by the exhaust gases to cool and solidify into spherical glassy particles called fly ash (Komatka et al., 1994).
UNDERSTANDING FLY ASH
Types of Fly Ash
As is the case with any raw material, varying the geographic source of fly ash may affect the properties and performance. There are two primary classes of fly ash used with cement: Class F and Class C. Class F fly ash is produced from burning older anthracite or bituminous coals with a low calcium content, CaO (Calcium hydroxide or quicklime, as it is commonly known) < 15%, and has no cementitious properties on its own. Class C fly ash is produced from burning younger lignite or sub-bituminous coals and are further sub-classed as intermediate calcium content (CI) with 15% < CaO < 20% and high calcium content (CH) with CaO > 20%. Importantly, as a result of the high CaO content, Class C fly ash will hydrate and harden on its own in the absence of a cementing agent or activator (Komatka et al., 1994).
How Fly Ash Works
When Portland cement and water are combined, compounds of calcium silicates (CS) and water react to form a calcium silicate hydrate (CSH) gel and calcium hydroxide (CH). The CSH is the binder while CH is not. By adding fly ash which contains reactive silica, however, the CH will react to form more CSH. Fly ash also acts as a microfiller between cement particles reducing permeability and bleed water (King 2005).
Fly Ash Advantages
While fly ash was once only a waste product, its use has steadily increased over the last 40 years. That said, still only 43.7% of fly ash was used in 2013 in the U.S. and the rest—approximately 30 million tons—was landfilled as waste (www.acaa-usa.org/Publications/Production-Use-Reports. 01/14). When it is utilized, however, it often realises a further environmental benefit by substantially reducing the CO2 emissions of concrete. Fly ash can also result in regulatory body issued incentives to related projects and facilities. This, coupled with the fact that it is cheaper at the production source than cement, often results in both environmental and economic benefits.
The use of fly ash in concrete also has several known physical advantages includ- ing the reduction of water demand, bleed water, heat of hydration, and permeability while also extending set times, increasing pumpability and, generally, the long term strength (King 2005).
As many of these benefits are also desirable in two-component annulus grouts, fly ash use in tunnelling is common. (Two-component grouts are also known as “bi- component” or “A/B type” grouts.) Annulus grouts, however, differ substantially from concrete in that they contain no aggregates and the water to cement ratio varies roughly between 2.0 and 3.0 versus 0.4 to 0.6 in concrete. The questions thus arises, do two- component annulus grouts see the same benefits from fly ash that concrete does?
Fly Ash Disadvantages
Apart from the performance of the grout itself, the logistics of obtaining and utilizing fly ash also need to be considered. Tunnelling projects are generally short term projects of intense activity executed within the tight physical boundaries of urban space. Consequently, these sites are often required to minimize and prioritize site activity. Using fly ash, however, requires a separate storage silo and conveyance system which may occupy valuable space and the benefit-cost ratio may be low for the additional equipment in a project with a timeline that is measured in months. Fly ash deliveries also require coordination with other site traffic and an awareness of the different raw materials. It has been the author’s experience that many sites using both fly ash and cement will, at some time during the project, place fly ash in the cement silo or vice versa causing downtime and economic loss.
The material savings from using fly ash may be muted by the added cost of transportation. As fly ash is produced by coal fired plants it will not be locally available if power generation is done by other methods, as is the case in the Pacific Northwest. Accordingly, as a result of both the transportation cost and increased demand in the concrete industry, the author has seen the delivered cost of fly ash within 10% of the cost of cement in several regions.
TESTING
Testing Methods
The laboratory procedures used for testing two-component grouts were previously developed by the author (Antunes 2012). It is important to note that these procedures include provisions for sample testing at various grout ages to capture any performance changes between the time the grout is first mixed and when it is ultimately injected into the annulus. Accordingly, the samples were tested at 1, 4, 24 and 48 hours as these times reflect the range of times that the grout is typically used within tunnelling projects.
It is also important to note that a colloidal mixer was used to mix the grout used for testing. It has been shown that colloidally mixed grouts achieve substantially higher compressive strengths and lower bleed water than grout mixed in traditional paddle type mixers (Reschke and Noppenberger 2011; Reschke 1998).
Ingredient Sources
The geographical source and type of fly ash are expected to affect the grout testing results. As transportation costs can be almost as much as the cost of the raw product, many project sites do not have the luxury of economically obtaining fly ash from different sources. The intent of this research is also to determine the impact that varying the source of fly ash can have on the annulus grout performance. As such, fly ash for the tests was sourced as follows:
- Control—Class F
- Northwest—Class F
- Southwest—Class F
- Northeast—Class C
- Southeast—Class C
All other ingredients were held constant:
- Cement—Type 1/GU
- Bentonite—Wyoming source
- Retarder— Pozzolith 100XR (BASF)
- Accelerator—Sodium Silicate N38
Mix Design
The mix design shown in Table 1 Test Results was used for all tests. It is based on a typical two-component grout with a 1 hour strength of 0.1 MPa and a 28 day strength of 3.5 MPa while being stable for at least 48 hours. (This mix design should not be used without testing with locally sourced materials as properties could vary substantially.) The intention was to provide a simple control mix to establish baseline parameters from which the effects of varying fly ash content could be studied. Five different fly ash/ cement ratios were tested for each of the five fly ashes sourced: 0:1 (0% fly ash), 1:3 (25% fly ash), 1:1 (50% fly ash), 3:1 (75% fly ash) and 1:0 (100% fly ash). Note that the 75% and 100% fly ash ratios are not typically used in practice but were included to capture the data extremes.
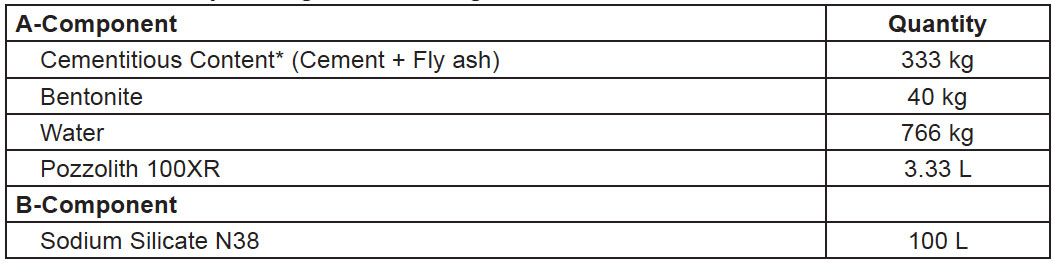
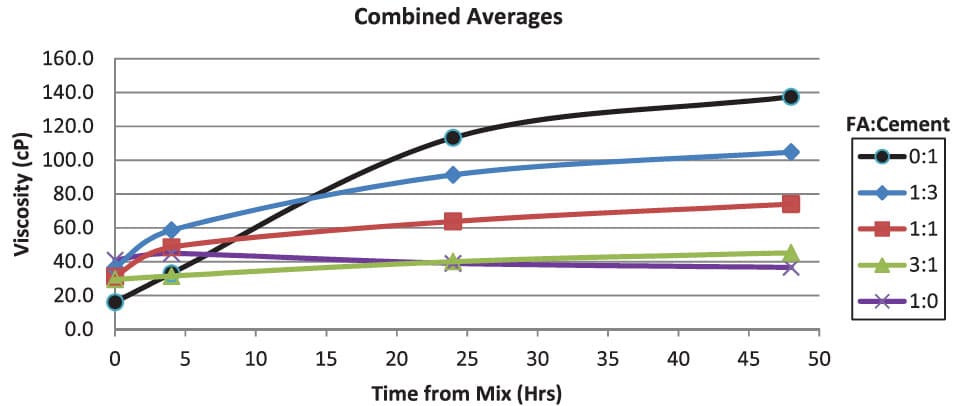
TEST RESULTS
Two-component annular grouts are typically mixed on surface and then pumped to the TBM via a steel pipe. Several mix properties need to be considered to allow the stabilized and then accelerated grout to be effective. These include:
- Viscosity
- Bleed
- Gel Time
- Compressive Strength (Antunes 2012).
Though the testing produced over 25 charts and numerous tables of data, the trends were remarkably similar between each fly ash tested. As such, the data was averaged to produce graphs that concisely indicate the trends observed.
Viscosity
The viscosity of the A-component is particularly important in determining pipeline specifications and surface pump pressures. A lower viscosity is preferred as it results in lower pump and pipeline pressures as well as lower power consumption.
Figure 1 shows the viscosity of the A-component grout with age and with varying fly ash contents. An important observation is that increasing the amount of fly ash in the mix lowered the mix viscosity. As length and diameter of tunnels are increasing— requiring higher grout volumes and pumping pressures—lower mix viscosities are a direct benefit to the grout conveyance system. Although increasing the retarder/stabilizer dose also reduces the viscosity, it also adds cost to the mix.
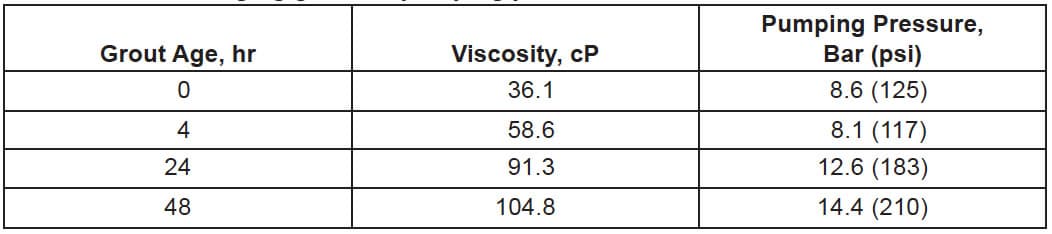
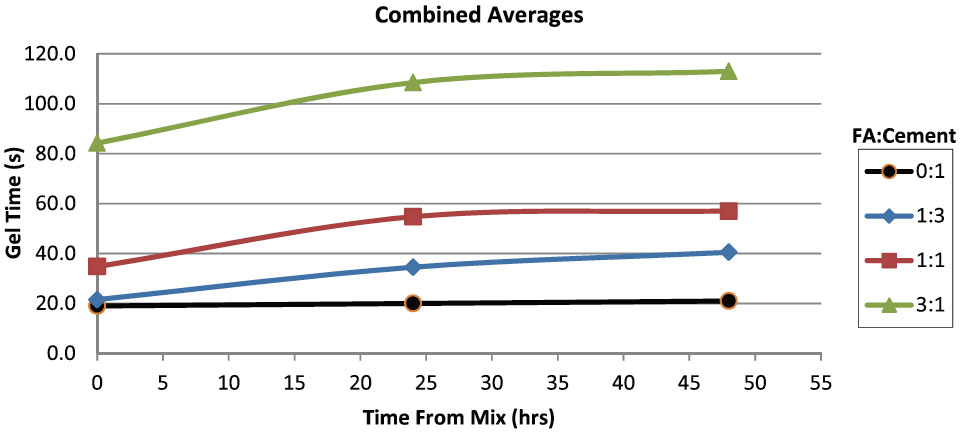
Of additional note is that all mixes had a relatively low viscosity when first mixed. However, as the stabilized grout aged, the viscosity increased in each case except for the 1:0 ratio which consisted of fly ash only (no cement). The practical implications of the effects of aging grout can be illustrated using the 1:3 ratio mix and the following assumptions:
- Pumping 15 m3/hr of grout
- 3″ Sch 40 steel pipeline
- 3 km long with no elevation change
- Grout specific gravity 1.23
Table 2 shows the theoretical pumping pressures in relation to the age of the grout. It is clear that as the A-component grout ages the pump pressure requirements climb substantially. This is an important factor to consider when selecting equipment for the project. It is also interesting to note that there is a drop in pressure between the 0 and 4 hour viscosities. This is due to the transition between laminar and turbulent flows in the pipeline. This transition will occur with any fluid and is a function of the pipe diameter, fluid velocity, and viscosity.
Gel Time
When the A and B components are combined, a gel starts to form in a short period of time (<40 s). This accelerated grout needs to remain fluid long enough to uniformly fill the annular space behind the tunnel segments without leaving voids. Conversely, the grout must also gel quickly enough to lock the segments in place and to resist being washed out by groundwater.
Figure 2 shows the effect of aging on grout gel time as well as the effect of fly ash content on gel time. There is a consistent trend that gel time increases with increased fly ash content and with the age of the grout.
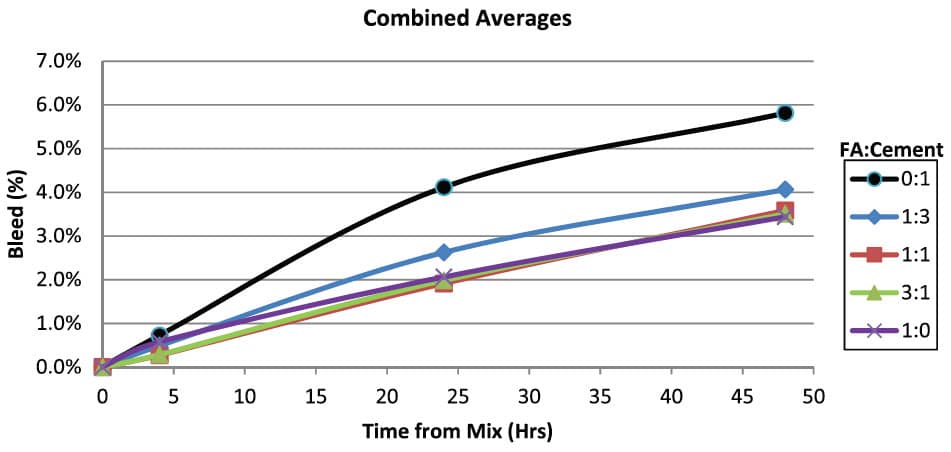
Based on the author’s experience, a gel time between 15 and 40 seconds is considered favourable, though this varies primarily on how many injection ports are being used on the TBM and the corresponding time required to fill the void. The results show that fly ash ratios up to 1:1 increased the gel time favourably. Gel time can also be affected by adjusting the B-component (also known as accelerator) dose. Contrary to general thought, however, increasing the B-component dose will actually increase your gel time though when it does gel, it will also result in higher early compressive strengths.
Bleed
The conveyance line delivering the A-component grout from the slurry plant to the TBM forms the critical link tying together grout production and TBM advance. Recognizing and minimizing bleed is necessary to reduce the risk of pipeline sedimentation and/or blockage. If the grout bleeds significantly, heavier particulates may begin to accumulate in the invert of the grout line and eventually reduce the cross sectional area of the pipe. This will lead to higher pumping and line pressures, lower flow rates and may ultimately completely occlude the pipe. As the high water/cement ratio of two-component grouts causes them to bleed excessively and rapidly, controlling bleed is critical and bentonite is the primary ingredient to accomplish this.
The effects of fly ash on bleed are shown in Figure 3. The 1:3 fly ash/cement ratio grout shows a substantial reduction in bleed as compared to the grout prepared with no fly ash. However, the addition of still more fly ash does little to further reduce the bleed. As bentonite dosages are generally low, the impact to the mix cost is relatively small. Even so, the use of fly ash can still benefit the mix by reducing bleed.
Strength
The most common performance specification for the grout, given by designers and contractors, is an early compressive strength requirement. Early strengths are needed to ensure the segments do not sink within the annulus once internally loaded by the TBM backup gantry and to ensure adequate load transfer between the segmental lining and the soil.
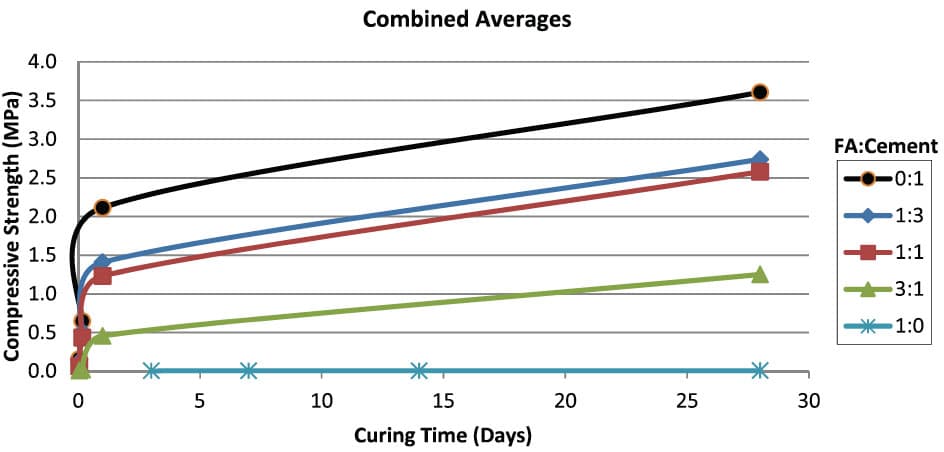
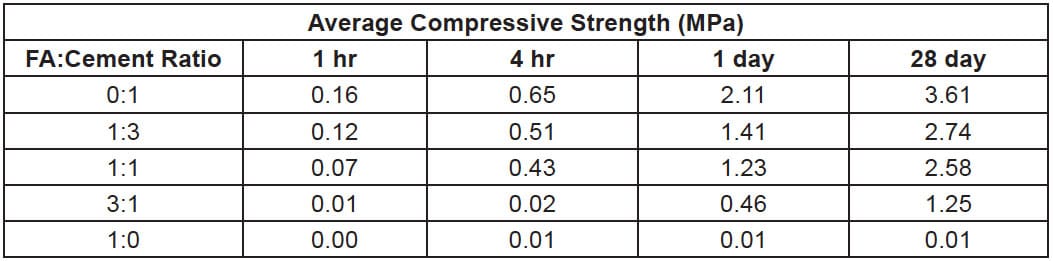
Figure 4 shows the effects of fly ash on the compressive strength of the accelerated grout and reveals a clear trend: as fly ash is increased, compressive strength decreases.
Table 3 contains the underlying data for Figure 4 to better quantify the effect that the fly ash ratio has on the compressive strength.
The compressive strength is clearly the property that suffers with the addition of fly ash. The early (i.e., <4 hr) strengths can be compensated for by increased accelerator to some degree. However, as the B-component is one of the most costly components of the mix, this option should be considered sparingly. If a higher long term strength is also needed, lowering the water/cement ratio is considered to be the best option. Unfortunately, reducing the water/cement ratio will increase viscosity and bleed and decrease the gel time. Testing is thus needed to optimize the project specific properties while reducing material costs.
Of additional interest is the effect that the age of the grout has on the compressive strength. After the A-component grout is mixed on surface, it can take anywhere from an hour to a few days before the grout is combined with the B-component. Figure 5 shows that as this wait time increases in grout containing fly ash, the 1 hour compressive strength actually declines whereas the opposite was true in grout with just cement. There were slight variances to this trend at the 1 and 28 day strengths but the overall trend indicates that this should be considered in mix design development.
CONCLUSIONS
The test results for two-component grouts utilizing fly ash from different geographic regions showed similar trends in data for each fly ash/cement ratio considered.
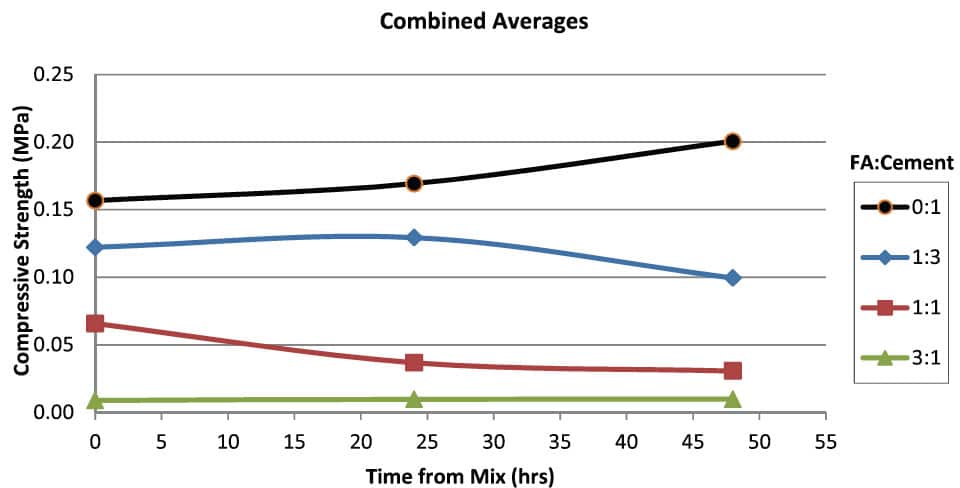
Viscosity and bleed saw pronounced decreases with fly ash addition which has positive implications to the design of the conveyance system. Gel time was increased which is favourable for the injection process. Compressive strength, however, decreased with the addition of fly ash. It has been the author’s experience that the grout benefits gained when using fly ash generally diminish when having to compensate for the reduced compressive strength. The testing conducted for this research did not specifically look at optimizing the mix design for a given compressive strength. Doing this may be beneficial for reference purposes but, in practice, would change from job to job.
REFERENCES
2013 Coal Combustion Product (CCP) Production & Use Survey Report. American Coal Ash Association, 2013. Web. 11 Jan. 2014. www.acaa-usa.org/Publications/ Production-Use-Reports.
Antunes, P. 2012. Early Age Testing Procedure of Two-Component Annulus Grouts. In North American Tunnelling Convention 2012 Proceedings, Indianapolis, IN, June 24–27. Englewood, CO: Society for Mining, Metallurgy and Explorations, Inc. (SME).
Derucher, K; Korfiatis, G; Ezeldin, A. 1994. Materials for Civil & Highway Engineers, 3rd ed. Englewood Cliffs, NJ: Prentice Hall.
King, B. 2005. Making better Concrete: Guidelines to Using Fly Ash for Higher-Quality, Eco-Friendly Structures. San Rafael, CA: Green Building Press.
Komatka, S; Kerhoff, B; Hooton,R. McGrath, R. 2010. Design and Control of Concrete Mixtures, 8th Canadian ed. Ottawa, ON: Cement Association of Canada.
Reschke, A. and Noppenberger, C. 2011. Brisbane Airport Link Earth Pressure Balance Machine Two Component Tailskin Grouting—A New Australian Record. In 14th Australasian Tunnelling Conference 2011, Auckland, New Zealand, March 8–10. Australasian Tunnelling Society.
Reschke, A. 1998. The Development of Colloidal Mixer Based CRF Systems. In Minefill ’98, Brisbane, Australia, April 14–16. Carlton, Victoria, Australia: The Australasian Institute of Mining and Metallurgy.